G. D. HESS
SEPTEMBER 24, 2020
I. INTRODUCTION
In this essay, G. Dale Hess dissects the scientific debate on the severity of the climatic effects of a regional nuclear war between India and Pakistan, namely the Global Nuclear Winter versus the No Nuclear Winter schools. He discusses the underlying assumptions of each school and lays out some questions for research before any definitive conclusion about the climatic effects of a limited nuclear war can be reached.
The essay may be downloaded in PDF format here.
G. Dale Hess was born in the United States where he studied atmospheric science. He came to Australia in 1970 and has worked in the area of Boundary-Layer Meteorology, which covers the physical, chemical and biological processes occurring in the lowest few kilometres of the Earth’s atmosphere. He retired from the Bureau of Meteorology 15 years ago. He is a former University Fellow at the University of Melbourne.
This essay is a working paper prepared for The 75th Anniversary Nagasaki Nuclear-Pandemic Nexus Scenario Project, October 31-November 1, and November 14-15, 2020, co-sponsored by Research Center for Nuclear Weapons Abolition, Nagasaki University (RECNA), the Nautilus Institute, Asia Pacific Leadership Network for Nuclear Non-proliferation and Disarmament.
Acknowledgements
The author would like to thank Richard Tanter, M. V. Ramana, Frank von Hippel and David Karoly for helpful discussions and reviewing the manuscript. Richard originally invited the author to work on this problem. The support and encouragement of Peter Hayes is also much appreciated as is the insightful comments of the anonymous reviewers
The views expressed in this report do not necessarily reflect the official policy or position of the Nautilus Institute. Readers should note that Nautilus seeks a diversity of views and opinions on significant topics in order to identify common ground.
This report is published under a 4.0 International Creative Commons License the terms of which are found here.
Banner image: effect of thermal radiation on building in US nuclear test, US DOD footage from Smithsonian here.
II. NAPSNET SPECIAL REPORT BY G. DALE HESS
THE IMPACT OF A REGIONAL NUCLEAR CONFLICT BETWEEN INDIA AND PAKISTAN: TWO VIEWS
SEPTEMBER 24, 2020
Abstract
The severity of climatic effects of a regional nuclear conflict between India and Pakistan, involving the use of a hundred Hiroshima-scale nuclear weapons, is contested between two groups; Mills, et al. (2014) conclude that a global Nuclear Winter would occur; Reisner, et al., (2018) conclude that No Nuclear Winter would occur. This paper discusses the different assumptions that lead to the two different conclusions. Specifically, it highlights the use of different fuel loading and different input methods for the amount and initial location of black carbon (BC) into the climate models, and discusses some underlying reasons for these different choices, including the question of what kind of fire will occur in the aftermath of a nuclear weapon being dropped on a densely populated city. The paper also briefly discusses some physical phenomena that have not been considered by either group and lays out some questions for research before any definitive conclusion about the climatic effects of a limited nuclear war can be reached.
The Problem
Could a regional conflict between two countries, say between India and Pakistan, which have far smaller arsenals in comparison to the Soviet Union and the United States in the 1980s, create climatic consequences severe enough to cause a global nuclear winter? This question is now being examined using the more sophisticated numerical models of the 21st century. The power of supercomputers has increased by a factor of more than 100 million since the 1980s (History-Computer, n.d.; Green, 2019). This power allows modellers to use sophisticated earth systems models, which include the atmosphere, ocean, land surface, land ice, sea ice, and associated physics, chemistry, and biology, to investigate the impact of a nuclear war.
We present the research from two groups who come to different conclusions. One group is Mills, et al. (2014) from the National Center for Atmospheric Research (NCAR), University of Colorado, and Rutgers University. The other group is Reisner, et al. (2018) from Los Alamos National Laboratory (LANL). LANL is where the world’s first nuclear weapons were designed as part of the Manhattan Project during World War II; today it is one of the largest scientific institutions in the world, conducting multidisciplinary research into many fields, including national security.
The two groups differ in their treatment of the source of black carbon (BC) aerosol (soot)[1] for the climate models, and the kind of fire which will occur in the aftermath of a nuclear weapon being dropped on a densely populated city. These differences lead Mills, et al., to conclude that a regional nuclear conflict would cause a global Nuclear Winter; Reisner, et al., find smaller climatic effects and conclude there would be No Nuclear Winter.
Climate model
Both groups utilise the NCAR Community Earth System Model (CESM1), which is a state-of-the-art, global climate model, configured with fully interactive ocean, land, land ice, sea ice, and atmospheric components (Hurrell et al., 2013). The aerosol and radiation components have been adapted for the treatment of BC (Toon, et al., 1988) and the chemistry module has been tailored to the middle and upper atmosphere (Kinnison, et al., 2007).
Model Set-up
In addition to using the same Community Earth Systems Model both groups use similar methods to prescribe the initial state of the atmosphere, ocean, and land surface. They perform a series of experiments (called an ensemble). Each experiment is conducted with slightly different initial values of the meteorological variables, i.e. the initial values are perturbed. The evolution of the system over time is sensitive to these small changes in the initial values, and the statistics of the differences in the simulations (i.e. in the members of the ensemble) will be a measure of the natural variability[2].
Masses of black carbon particles are created by the fires resulting from the use of nuclear weapons. The procedure is for each research group to conduct a number of simulations with BC particles added where each simulation has slightly different initial conditions. They also conduct simulations with the same variation in initial conditions as the previous simulations but without BC added. This latter set of simulations is called the ‘control’ runs. The difference between the simulations with BC added and the control runs will indicate the impact on the climate. During the simulations, the greenhouse and other gas constituents are allowed to change according to the Representative Concentration Pathway (RCP4.5) scenario[3].
Coagulation is neglected in the simulations which means that the size of the BC particles (50 nm (50 × 10-9 m) or 100 nm radius) stays the same throughout the simulation. The BC particles are assumed to be hydrophilic (they attract water) from the start and hence are subject to rainout.
Mills, et al., and Reisner, et al., both consider a nuclear war in which each side detonates 50 15-kt (equivalent to 15,000 tons of TNT) nuclear weapons on leading population centres in South Asia. By today’s standards a 15-kt yield is considered to be a relatively small bomb; it is roughly the yield of bomb dropped on Hiroshima. The groups differ in how they implement the impact of the denotations into their models.
a. Mills, et al.
For both groups, there is limited data available on fuel loading in urban centres in developing countries. Toon, et al. (2007), make estimates based on population density and databases for the developed world and reduce them by multiplying the estimates by a factor of 0.5 to apply to the developing world, based on fuel load data currently available. The amount of BC produced is estimated by considering population density, the fuel burden per capita, the area impacted by fires, the fuel loading in different category types, the fraction of fuel assumed to burn following nuclear ignition, the elemental carbon (soot) emissions factor, a conversion factor to convert urban data from the developed to the developing world, and the loss due to rainout. The area burned by a 15-kt bomb is assumed to be 13 km2 (the same as Hiroshima).
Based on the study of Toon, et al., Mills, et al., add 5 Tg (5 × 1012 g) BC to their model through a parameterisation method[4]. To the initial atmospheric conditions, Mills, et al., insert BC in a constant mass mixing ratio of 1.38 × 10?6 kg/kg air between 300 hPa[5] (~9 km) and 150 hPa (~13.5 km) in a horizontal region spanning 50 adjacent model columns roughly covering each of India and Pakistan. The height of the region of the injection is in the upper troposphere[6] and is chosen to coincide with the simulations of the smoke plume arising from the bombing of the city of Hamburg during World War II (Penner, et al., 1986) and the 2001 Chisholm wildfire in Alberta, Canada (Luderer, et al., 2006). The fraction of BC removed from the atmosphere by rain processes is chosen to be 20%, based on laboratory and wildfire observations (e.g. Turco, et al., 1990).
b. Reisner, et al.
Reisner, et al. (2018) determine the vertical distribution of BC by simulations of the fireball using the HIGRAD-FIRETEC model[7] (e.g. Fierro & Reisner, 2010).
The nuclear explosion is modelled as an airburst at twice the fallout-free height (324.16 m)[8]. No secondary ignitions, such as the gas line breaks that occurred in Hiroshima, are considered. The combustion chemistry is not modelled. Instead, the BC production is parameterised to be inversely proportional to oxygen depletion, that is, it is assumed that all the carbon in the fuel participated in the reaction and was turned into BC. If the buildings are composed of wood, the FIRETEC model represents the burning of the entire buildings, but not otherwise; examination of data from Google Earth by Reisner et al. suggests that cities in India and Pakistan are constructed primarily of concrete. In addition, because LANL is a nuclear weapons laboratory, Reisner et al. are reluctant to choose a foreign city as a nuclear target. Hence a ‘generic suburb’ of Atlanta, Georgia, is selected as a target where building, vegetation and fuel loading data were available. A target area of 10 km × 10 km is specified for ground effects. This is a much larger target area than used by Mills, et al., but what is more important is the fuel loading is much less than adopted by Mills, et al.
The vertical distribution of BC emissions is added from 11 minutes of fire simulation taken at 1-minute intervals. The BC emissions are added to three grid columns between roughly 27.5°N–67.5°E and 31.3°N–72.5°E over Pakistan and to three grid columns centred around 27.5°N–80°E over India. The difference in methodology, regarding timing and horizontal location of BC emissions, here to that of Mills, et al., is not of lasting significance.
The total magnitude of the BC emitted in the model ranges from 3.68 Tg to 3.72 Tg with the bulk of it at heights below 12 km. Thus, the emissions are less than employed by Mills, et al. and the peak concentrations are at lower heights.
Worst Case
In order to assess the climatic impact both groups attempt to simulate the worst-case fire that would arise from the nuclear conflict. Mills, et al., assume that the worst case is a firestorm (e.g. as occurred at Hiroshima with its largely wooden buildings[9]) where many fires merge into single column. The criteria for a firestorm, based on the mass fires of World War II, are (Glasstone and Dolan, 1977):
- burning area ? ~ 1.3 km2
- half the structures in the area are on fire simultaneously
- a fuel load ? 4 g cm-2
- ambient winds < 3.6 m s-1, the fire is stationary
This fuel-load requirement for a firestorm is met for all of the Indian and Pakistani high-density cities considered in this present scenario (Toon, et al, 2007).
The worst case for Reisner, et al., is determined in a different way. The HIGRAD-FIRETEC model provides a rubble or no rubble option, and Reisner, et al., concentrate on the no rubble option. The presence of rubble inhibits fire effectiveness in the centre region of the blast because of a lack of oxygen, leaving a donut ring of fire which is unable to support a firestorm. Once the shock wave from the blast arrives, buildings could collapse. For buildings which do not collapse, fires caused by the nuclear-weapon fluence could lead to the burning of the building and its contents, if it is made of flammable material such as wood. In contrast, in collapsed buildings composed of cement or other non-flammable material, fires would likely become quickly oxygen?starved and begin to smoulder. The region of maximum fluence and shock impact would lead to collapsed buildings and smouldering fires in the central area with a circular ring of fires at the boundary.
Reisner, et al., specify a dry, stable atmosphere. Hence, they do not consider pyro-convection[10] which may increase the buoyancy of the plume or it may reduce BC concentrations through precipitation scavenging, i.e. where rainfall removes the BC particles. The worst case they consider is mass conflagration fire[11] (e.g. as produced by conventional bombing in Tokyo). They have 400 000 points of ignition which merge into one mass fire where strong inwardly directed winds develop and there is little fire spread.
Results for Mills, et al.
Black carbon arising from the fires produces two significant effects. It not only cools the Earth’s surface but also severely heats the region near the stratopause (50 km – 60 km height) (with excess temperatures of more than 80 K) which accelerates catalytic loss cycles for ozone. Ozone plays an important role in absorbing harmful ultra-violet radiation and protecting life on Earth.
Mills, et al., find the smoke plume penetration into the stratosphere results in massive global ozone losses of 20%–50% over populated areas; these losses are on a scale never previously observed and would result in damage to human health, agriculture, and ecosystems. These losses would accompany the coldest average surface temperatures in the last 1000 years, i.e. Nuclear Winter. The effects could last more than two decades and could trigger a global nuclear famine.
The upper layer of the ocean experiences a prolonged cooling that creates a significant long-lived thermal deficit. Because of the large heat capacity of the ocean, this thermal deficit maintains reduced surface temperatures for decades. The surface cooling is reflected in an increased extent of sea ice. In the Arctic, a maximum increase of 10%–25% is reached in years 4–7. In the Antarctic, the maximum increase in sea ice extent is 20%–75% obtained in years 7–15.
The model shows that killing frosts would reduce the length of the average growing season up to 40 days throughout the world’s agricultural zones in years 2 through 5.
Results for Reisner, et. al.
Reisner, et al. find that the average height of the fireball plume after 3.5 minutes of simulation is 13 km where it begins to stabilise in altitude. This result is consistent with the empirical result of 10–13 km for a 15-kt explosion found by Glasstone and Dolan (1977). Initial updrafts in the lower troposphere are of order 100 m s-1 but only a relatively small amount of BC makes its way into the stratosphere during the course of the simulation. Most of the BC is below 9 km where 78% is rained out within the first two months. In contrast, Mills, et al. (2014) found that 60% – 70% of the BC mass is transported to the stratosphere within the first couple of weeks.
Reisner, et al., conduct simulations with an initial distribution of BC concentrations as described by Mills, et al., to compare with the simulations where the BC distributions are generated by the HIGRAD-FIRETEC model. Figure 1 shows the e-folding time scale[12] for BC mass reduction is 4.2 years for the Reisner, et al., model and 8.4 years for the Mills, et al., BC distribution. The HIGRAD-FIRETEC result is close to the results of Robock, et al. (2007), Mills, et al. (2008) and Stenke, et al. (2013) for BC mass of 1 Tg, i.e. for a BC mass of one-fifth of the initial amount used by Mills, et al. (2014).
Figure 1. The time variation of BC mass for the HIGRAD-FIRETEC model (solid blue line) and for the simulation with an initial BC distribution as in Mills et al. (2014) (solid red line). The dashed lines represent the mass at heights above 200 hPa (~12 km). (After Reisner, et al., 2018).
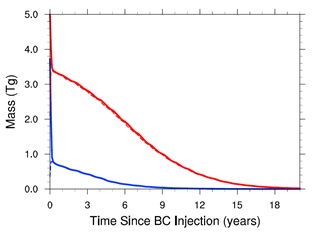
Figure 2 shows the difference in the ensemble mean values in the globally averaged surface temperature of the atmosphere, net solar radiation flux at surface and the precipitation rate when the simulations included BC and when BC was absent. The shaded areas are the sum of standard deviations from the ensemble with BC present plus the one without BC as calculated by Reisner, et al. They represent the range of normal variability. If the solid lines lie within the shaded areas the differences may not be distinguished from normal variability. For temperature, the deviations from normal variability occur only for the first few years. The greatest deviation is seen in the net solar radiation results where the effects last for 5 years.
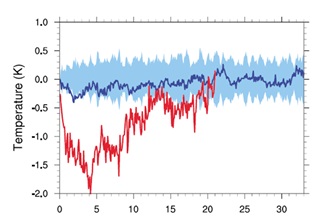
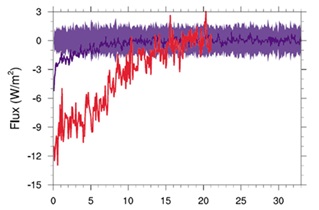

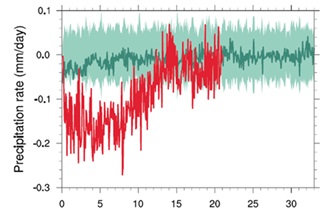
Figure 2. The time variation of the global monthly average anomalies for the near-surface atmospheric temperature (top), net solar radiation flux (middle), and precipitation rate (bottom). The red lines are corresponding results using the same BC source as in Mills et al. (2014). Shading represents the normal range given by the sum of the ensemble standard deviations relative to the ensemble means. Ensemble anomalies represent the departure from the respective control simulation ensembles. (After Reisner, et al., 2018).
The red lines indicate the Reisner, et al., results based on the initial BC distribution of Mills, et al. These show much larger deviations from the normal range and they last for a decade or longer because the plume has lofted high into the stratosphere where the residency time is longer.
Discussion
Both Mills, et al. (2014) and Reisner, et al. (2018) consider the same regional nuclear war scenario, and both use the same Community Earth System Model. They differ in their treatment of the source of black carbon. Mills, et al., conclude that a Nuclear Winter would occur after the war which would have a severe global climatic impact lasting more than a decade. Reisner, et al., find there would be a small global climatic impact lasting a few years and they conclude there would be No Nuclear Winter.
Criticism of Reisner, et al. (2018)
Robock, et al. (2019) criticise the study of Reisner, et al., for not considering a firestorm as produced in Hiroshima, Hamburg and Dresden in World War II, which Robock, et al., see as the worst case. Without Reisner, et al., demonstrating their model can produce a firestorm, Robock, et al., find it difficult to interpret the impact of the Reisner, et al., results on the climate. Their fuel loading from suburban Atlanta is too small to generate a firestorm. Robock, et al., calculate the Reisner, et al., fuel loading in the 13 km2 target area to be 0.14 g cm-2 and in the 10?km × 10?km domain of the Reisner, et al., model to be 0.91 g cm-2; both values are well below the threshold needed for a firestorm. The fuel load assumed by Reisner, et al. is more than an order of magnitude less than any of the 100 urban areas of Pakistan or India considered by Robock et al. (2007) and Mills et al. (2014).
Secondly, they criticise Reisner, et al., for choosing a stable, dry atmosphere which could suppress the development of the fire, and not considering pyro-convection and the release of latent heat which eliminates a major source of buoyancy. Pyro-convection lofted the smoke from the British Columbia 2017 wildfires into the stratosphere (Yu, et al., 2019).
Thirdly, they criticise Reisner et al. for simulating a fire duration of only 40 minutes. In contrast, the firestorms at Hiroshima and Hamburg, and the mass conflagration fire at Tokyo during World War II lasted many hours.
Robock, et al., conclude that the case presented by Reisner et al. is not typical of the conditions that would be expected in a nuclear war between India and Pakistan.
Reply by Reisner, et al. (2019)
Reisner, et al. (2019) respond to the criticism of Robock, et al. (2019) of low fuel loading by performing two new simulations. One simulation had no rubble and a fuel loading of 4 g cm-2 which meets the firestorm criterion, and the other simulation has constant fuel and fuel loading of 72 g cm-2 which is near the upper range of values of fuel loading calculated by Toon, et al., (2007) (12.6 to 94.5 g cm-2) for the top 50 urban targets in India and Pakistan.
Reisner, et al., find that increasing the fuel load for the no rubble case from 1 g cm-2 in the original study to 4 g cm-2 results in a BC mass increase by a factor of ~2. With a constant fuel load of 72 g cm-2, the BC increase is only a factor of ~6. Thus, the simulations show there is a nonlinear relationship between increasing fuel load and atmospheric BC content. This is interpreted as being a result of inadequate oxygen availability due to intense combustion and limited resupply by entrainment of ambient air. These factors tend to saturate BC production at high fuel loads.
Reisner, et al., state, based on observations of biomass burning, they overestimated the amount of BC mass emitted by a factor of 10–100 by assuming all fuel is converted to BC. They admit that they simulated a mass conflagration rather than a firestorm, but contend that a firestorm is unlikely to occur, because of the high density of concrete buildings in urban settings in India and Pakistan and because concrete buildings will not burn readily during a fire. Because concrete buildings are easily destroyed by the blast wave, they would produce a lot of rubble that would further inhibit the fires that would develop. Furthermore, even though their simulations are not representative of Indian or Pakistan urban areas, Reisner, et al. contend that they do represent what is expected after an in-air detonation of a nuclear device. The simulations produce a massive fire from a very large number of simultaneous ignitions, an intense initial fire pulse that creates strong upward motions and strong inward winds which limits the horizontal spread.
While the full duration is not modeled, Reisner, et al., argue that the primary atmospheric response from an in-air nuclear detonation is the rapid burning of the fine fuels (e.g. paper). The simulations show rapid burning of the fine fuels which limits combustion by reducing the available oxygen and also transports a large quantity of heat upwards which stabilises the upper atmosphere. Both processes act to limit fire activity and BC production. Hence most of the BC emissions occur in a relatively short period of time. The burning or smouldering of the thicker fuels (e.g. furniture) can continue for longer periods, but less BC is injected into the upper atmosphere.
Reisner, et al., admit they didn’t include pyro-convection in their simulations but argue that the release of latent heat may or may not lead to enhanced smoke lofting. The effect of increased buoyancy may be countered by precipitation scavenging which removes BC. The removal may be greater than the 20% assumed by Mills, et al.
Reisner, et al., conclude that without rigorous analysis, similar to what they have initiated, discussions regarding nuclear winter plausibility are premature.
Uncertainties
There are many uncertainties inherent in this type of modelling. There is uncertainty about the fuel type and fuel load[13]. This information is crucial in determining the type of fire that would result, a firestorm or a mass conflagration.
There are uncertainties about the role of pyro-convection in plume rise and the accompanying rainout during plume rise. Pyro-convection modelling, including fractal coagulation (aggregations in complex geometric shapes), and plume rise verification based on field data for both dry and wet conditions need to be performed.
Further research needs to be performed to determine the emission factors for BC and POM and the BC/POM ratio and the oxidation pathways for organics. Experiments are needed to determine whether modern building materials will burn, and how to upscale results on small material samples to the burning of cities. In addition, the interaction between fire and the shock wave needs to be better understood.
To produce a significant climatic effect Reisner (2019) estimates that a minimum of 50 – 100 cities within a region must suffer nuclear attack, as described above. The key factor is how much BC reaches the upper troposphere and lower stratosphere[14].
The outcome of nuclear war will be horrific for human security under any scenario. The outcome could be far worse than presented here.
By 2025 India and Pakistan may have 400 to 500 nuclear weapons. If nuclear war breaks out between these countries, and India uses 100 strategic weapons to attack population centres and Pakistan uses 150 weapons, Toon, et al. (2019) predict that fatalities could reach 40 to 125 million people and the resulting fires could release 16 to 36 Tg BC into the upper troposphere.
For a nuclear conflict between the United States and Russia up to 150 to 180 Tg BC could be lofted into the upper troposphere (Coupe, et al., 2019).
Serious scientific study of plausible scenarios and their consequences is urgently needed.
Both strategic/military policy and peace/disarmament movement policy must be based on the best possible evidence rigorously and openly assessed and then used with any caveats or acknowledgement of limitations of our understanding, and without groundless exaggeration, as the basis for informing global public in accessible language and formats.
Reducing the uncertainties
Jon Reisner (Reisner, 2019) gave a seminar at the National Center for Atmospheric Research on November 12, 2019 in which he discussed the need to reduce the uncertainties and appealed to the community for help to do this. Work is underway at LANL to upgrade HIGRAD-FIRETEC to run faster, and to include detailed chemical kinetics (the formation of black carbon), probability density functions for the mean temperature and its variation within a grid cell, pyro-cumulus formation and the release of latent heat. Validation tests with other fire models and field data are being carried out, as well as tests on modern building materials to see if they will burn. Reisner’s recent simulations have been unable to produce a firestorm over Los Angeles or Chicago with a 100-kt detonation.
Conclusions
Mills, et al. (2014) and Reisner, et al. (2018) have each studied the climatic impact of a regional war between India and Pakistan but have arrived at different conclusions. Mills, et al., predict such a war would initiate a global Nuclear Winter that would last for more than a decade. Reisner, et al., predict there would be some climatic effects in the first five years, but that they would be insignificant and there would be No Nuclear Winter. The reason for the difference in the two studies lies in the different way the two groups treat the source of black carbon in their models. It highlights the uncertainties in present understanding of this important problem and model inadequacies. Research is now under way to reduce these uncertainties, but the problems are complex, and solutions will require cooperation from the larger scientific community[15]. However, until these problems are resolved, from a disarmament point of view, the precautionary principle is, in situations of such high stakes involving millions of deaths, there is an obligation to assume a worst-case scenario. Such a scenario would include the possibility that a limited nuclear conflict could cause a Nuclear Winter by leading to a broader nuclear conflict.
References
Coupe, C. G. Bardeen, A. Robock, and O. B. Toon (2019). Nuclear winter responses to nuclear war between the United States and Russia in the Whole Atmosphere Community Climate Model Version 4 and the Goddard Institute for Space Studies ModelE, J. Geophys. Res.: Atmos. 124, 8522–8543.
Fierro, A., and J. M. Reisner (2010). High-resolution simulation of the electrification and lightning of Hurricane Rita during the period of rapid intensification, J. Atmos. Sci., 68, 477–494.
Glasstone, S., and P. J. Dolan (1977). The Effects of Nuclear Weapons, Third edition, United States Department of Defense, and Energy Research and Development Administration.
Green, T. (2019). 10 of the world’s fastest supercomputers, Networkworld, 18 November 2019. https://www.networkworld.com/article/3236875/embargo-10-of-the-worlds-fastest-supercomputers.html#slide5.
History-Computer (n.d.). Cray Supercomputer. https://history-computer.com/ModernComputer/Electronic/Cray.html.
Hurrell, J. W., M. M. Holland, P. R. Gent, S. Ghan, J. E. Kay, P. J. Kusher, J.-F. Lamarque, W. G. Large, D. Lawrence, K. Lindsay, W. H. Lipscomb, M. C. Long, M. Mahowald, D. R. Marsh, R. B. Neale, P. Rasch, S. Vavrus, M. Vertenstein, and D. Bader (2013). The community earth system model: A framework for collaborative research, Bull. Am. Meteorol. Soc., 94, 1339–1360. doi:10.1175/BAMS-D-12-00121.1.
Kinnison, D. E., G. P. Brasseur, S. Walters, and R.R. Garcia (2007). Sensitivity of chemical tracers to meteorological parameters in the MOZART-3 chemical transport model, J. Geophys. Res., 112, D20,302. doi:10.1029/2006JD007879.
Luderer, G., J. Trentmann, T. Winterrath, C. Textor, M. Herzog, H. F. Graf, and M. O. Andreae (2006). Modeling of biomass smoke injection into the lower stratosphere by a large forest fire (Part II): Sensitivity studies, Atmos. Chem. Phys. Discuss., 6, 6081–6124.
http://www.atmos-chem-phys-discuss.net/6/6081/2006/
Mills, M. J., O. B. Toon, R. P. Turco, D. E. Kinnison, and R. R. Garcia (2008). Massive global ozone loss predicted following regional nuclear conflict, Proceedings of the National Academy of Sciences of the United States of America, 105(14), 5307–5312. https://doi.org/10.1073/pnas.0710058105
Mills, M. J., O. B. Toon, J. L. Taylor, and A. Robock (2014). Multidecadal global cooling and unprecedented ozone loss following a regional nuclear exchange, Earth’s Future, 2, 161–176. https://doi.org/10.1002/2013EF000205
Penner, J. E., L. C. Hassleman Jr., and L. L. Edwards (1986). Smoke plume distributions above large-scale fires: Implications for simulations of “nuclear winter”, J. Clim. Appl. Meteorol., 25, 1434–1444.
Reisner, J. (2019). Climate impact of a regional nuclear weapons exchange: Improving the source assessment. Seminar at the National Center for Atmospheric Research, November 12, 2019.
Reisner, J., G. D’Angelo, E. Koo, W. Even, M. Hecht, E. Hunke, D. Comeau, R. Bos, and J. Cooley (2018). Climate impact of a regional nuclear weapons exchange between India and Pakistan: An informed assessment based on high?fidelity source calculations, J. Geophys. Res.: Atmos., 123, 2752–2772. https://doi.org/10.1002/2017JD027331
Reisner, J., E. Koo, E. Hunke, and M. Dubey (2019). Reply to Comment by Robock et al. (2019) on “Climate impact of a regional nuclear weapons exchange: An improved assessment based on detailed source calculations”, J. Geophys. Res.: Atmos., 124, 12,959–12,962. https://doi.org/10.1029/2019JD031281
Robock, A., L. Oman, G. L. Stenchikov, O. B. Toon, C. G. Bardeen, and R. P. Turco (2007). Climatic consequences of regional nuclear conflicts, Atmos. Chem. Phys., 7, 2003–2012. doi:10.5194/acp-7-2003-2007.
Robock, A., O. B. Toon, and C. G. Bardeen (2019). Comment on “Climate impact of a regional nuclear weapons exchange: An improved assessment based on detailed source calculations” by Reisner et al., J. Geophys. Res.: Atmos., 124, 12,953–12,958. https://doi.org/10.1029/2019JD030777
Stenke, A., C. R. Hoyle, B. Luo, E. Rozanov, J. Gröbner, L. Maag, S. Brönnimann, and T. Peter (2013). Climate and chemistry effects of a regional scale nuclear conflict, Atmos. Chem. Phys., 13, 9713–9729. doi:10.5194/acp-13-9713-2013.
Sunilkumar, S. V., A. Babu, and K. Parameswaran (2013). Mean structure of the tropical tropopause and its variability over the Indian longitude sector, Climate Dynam., 40, 1125–1140.
Toon, O. B., R. P. Turco, D. Westphal, R. Malone, and M. S. Liu (1988). A multidimensional model for aerosols: Description of computational analogs, J. Atmos. Sci., 45, 2123–2143. https://doi.org/10.1175/1520-0469(1988)045<2123:AMMFAD>2.0.CO;2
Toon, O. B., R. P. Turco, A. Robock, C. Bardeen, L. Oman, and G. L. Stenchikov (2007). Atmospheric effects and societal consequences of regional scale nuclear conflicts and acts of individual nuclear terrorism, Atmos. Chem. Phys., 7, 1973–2002.
Toon, O., C. Bardeen, A. Robock, L. Xia, H. Kristensen, M. McKinzie, R. Peterson, C. Harrison, N. Lovenduski, and R. Turco (2019). Rapidly expanding nuclear arsenals in Pakistan and India portend regional and global catastrophe, Sci. Adv., 5. eaay5478. 10.1126/sciadv.aay5478.
Turco, R. P., O. B. Toon, T. P. Ackerman, J. B. Pollack, and C. Sagan (1990). Climate and smoke: An appraisal of Nuclear Winter, Science, 247, 166–176.
Yu, P., O. B. Toon, C. G. Bardeen, Y. Zhu, K. H. Rosenlof, R. W. Portmann, T. D. Thornberry, R.-S. Gao, S. M. Davis, E. T. Wolf, J. de Gouw, D. A. Peterson, M. D. Fromm, and A. Robock (2019). Black carbon lofts wildfire smoke high into the stratosphere to form a persistent plume, Science, 365(6453), 587–590. https://doi.org/10.1126/science.aax1748
III. ENDNOTES
[1] Black carbon (BC, soot): Burning carbon without sufficient oxygen to allow complete combustion creates black carbon or soot. This is a form of elemental carbon, i.e. it is inorganic carbon in an amorphous form. BC emissions are important emissions from the fires because they absorb solar radiation; the resultant heating gives buoyancy to the plume. If the plume reaches the stratosphere (above the region of rainout which occurs in the lowest layer, the troposphere) the particles can block solar radiation and create cooling at the Earth’s surface for years. In addition to BC emissions, there may be significant amounts of particulate organic matter (POM) emitted from the fires. Organic carbon comes from living organisms and in most cases is associated with hydrogen. Organic carbon particles tend to reflect rather than absorb solar radiation.
[2] Both Mills, et al., and Reisner, et al., started their simulations on 1 January 2013. Mills, et al. also conducted simulations starting on 15 May, as was done by Robock et al. (2007) and Stenke et al. (2013). Mills, et al., found that the different season did not significantly affect the stratospheric distribution or climatic impact of the BC.
[3] A Representative Concentration Pathway describes future climate conditions by prescribing the evolution of the greenhouse gas concentrations over time. In RCP4.5 emissions peak about the year 2040 and then decline.
[4] The term parameterization refers to a simplified process used to replace a process that is too small-scale or complex or which requires too much computer time to be physically represented in the model.
[5] hPa stands for hectapascal, a unit of pressure. 1013.25 hPa is the value of mean sea-level atmospheric pressure.
[6] For latitudes from the Equator to 35 oN in the area of India and Pakistan, the troposphere extends from the ground to 16 – 19 km (Sunilkumar, et al., 2013). The height of the tropopause decreases going toward the poles. At the latitude of Hamburg, for example, it is at approximately 11 km, which makes plume penetration into the stratosphere easier.
[7] HIGRAD-FIRETEC: HIGRAD is a hydrodynamics model which solves the compressible Navier-Stokes equations; FIRETEC is a physics-based fire dynamics model; together they provide the source input for the amount and location of black carbon for Reisner, et al. (2018).
[8] At or above the fallout-free height the heat of the fireball creates a suspension of particles 10 nm to 20 ?m
(10-6 m) in diameter; there are no large particles because explosion debris from the surface does not reach this height and thus radioactive fallout is not an issue. Mills, et al., modelled their study on Hiroshima where the bomb was detonated at 600.46 m height.
[9] During World War II firestorms were created in Hiroshima by an atomic bomb, and in Hamburg and Dresden through incendiary conventional bombs. The wood fuel loading in Hamburg, for example, was estimated to vary between 12 g cm-2 and 47 g cm-2 depending on building density in the block (Toon, et al., 2007). These loadings meet the fuel-load criterion for a firestorm. Because the bombing was non-nuclear, shock waves creating rubble to inhibit the fires were limited. A firestorm did not develop at Nagasaki, probably due to the uneven terrain there.
[10] Pyro-convection is a cumulonimbus weather system created by the fire. As the plume rises in the cloud, it cools and condensation and freezing occur, which releases latent heat and creates buoyancy and further lofting of the plume. Pyro-convection can also reduce BC concentrations through rainout. If the fire diameter > atmospheric scale (~ 10 km) deep penetration of troposphere and lower stratosphere occurs by pyro-convection. For smaller fires, the plume ascent due to pyro-convection is limited to the middle and upper troposphere (Toon, et al., 2007).
[11] A mass conflagration fire is a large-scale fire arising from many different ignition points whereas a firestorm is a single column fire. The smoke from both types of fires can reach the stratosphere when pyro-convection is present. Mills, et al., parameterize pyro-convection; Reisner, et al., do not consider pyro-convection.
[12] The time required for the BC mass to reduce by a factor of e, where e is the base of the natural logarithm, and has a value of ~2.71828, is called the e-folding time scale.
[13] In addition to examination of Google Earth images of cities, on-site building surveys of combustible cladding /insulation and other materials both inside and outside buildings are needed.
[14] The amount of BC lifted to the upper troposphere and lower stratosphere depends on many factors, e.g. the amount and type of fuel available, the amount of rubble produced, atmospheric conditions, height of detonation, etc., not solely on the number of bombs.
[15] Support for this research may be forthcoming. The House of Representatives version of the National Defense Authorization Act for Fiscal Year 2021, currently before the US Congress, would require a National Academies study on the atmospheric effects of nuclear war.
IV. NAUTILUS INVITES YOUR RESPONSE
The Nautilus Asia Peace and Security Network invites your responses to this report. Please send responses to: nautilus@nautilus.org. Responses will be considered for redistribution to the network only if they include the author’s name, affiliation, and explicit consent.